Microsoft word - tepper.doc
The FASEB Journal express article10.1096/fj.03-0847fje. Published online June 18, 2004.
Electromagnetic fields increase in vitro and in vivo
angiogenesis through endothelial release of FGF-2
Oren M. Tepper, Matthew J. Callaghan, Edward I. Chang, Robert D. Galiano, Kirit A. Bhatt, Samuel Baharestani, Jean Gan, Bruce Simon, Richard A. Hopper, Jamie P. Levine, Geoffrey C. Gurtner The Laboratory of Microvascular Research and Vascular Tissue Engineering, New York University School of Medicine, New York, NY 10016
Oren M. Tepper and Matthew J. Callaghan contributed equally to this work.
Corresponding author: Geoffrey C. Gurtner, New York University Medical Center, Institute of Reconstructive Plastic Surgery, TH-169, 550 First Ave., New York, NY 10016. E-mail:
[email protected]
Pulsed electromagnetic fields (PEMF) have been shown to be clinically beneficial, but their mechanism of action remains unclear. The present study examined the impact of PEMF on angiogenesis, a process critical for successful healing of various tissues. PEMF increased the degree of endothelial cell tubulization (sevenfold) and proliferation (threefold) in vitro. Media from PEMF cultures had a similar stimulatory effect, but heat denaturation ablated this activity. In addition, conditioned media was able to induce proliferative and chemotactic changes in both human umbilical vein endothelial cells and fibroblasts, but had no effect on osteoblasts. Angiogenic protein screening demonstrated a fivefold increase in fibroblast growth factor β-2 (FGF-2), as well as smaller increases in other angiogenic growth factors (angiopoietin-2, thrombopoietin, and epidermal growth factor). Northern blot analysis demonstrated an increase in FGF-2 transcription, and FGF-2 neutralizing antibody inhibited the effects of PEMF. In vivo, PEMF exposure increased angiogenesis more than twofold. We conclude that PEMF augments angiogenesis primarily by stimulating endothelial release of FGF-2, inducing paracrine and autocrine changes in the surrounding tissue. These findings suggest a potential role for PEMF in therapeutic angiogenesis.
Key words: therapeutic angiogenesis • growth factors
lthough controversial, electromagnetic forces are believed to play a role in the normal repair of human tissues. The therapeutic efficacy of various forms of electrical
A stimulation, including capacitative coupling, direct current, combined magnetic fields,
and pulsed electromagnetic fields (PEMF), have been intensely investigated over the past 30 years (1, 2). The therapeutic effects of PEMF were first demonstrated in bone by Basset and colleagues (1), whose reports led to clinical trials and widespread commercial availability. Subsequently, PEMF has been demonstrated in blinded trials to be a safe and effective means of treating nonhealing bone fractures (3, 4)
(page number not for citation purposes)
Despite the clinical evidence demonstrating PEMF to be an effective treatment modality in bone healing, its mechanism of action is unknown. PEMF is able to up-regulate several cytokines that are important in promoting osteoblast differentiation during fracture repair, including bone morphogenetic proteins 2 and 4 and transforming growth factor-β (5, 6). However, more direct barometers of osteoblast function such as collagen synthesis, proliferation, alkaline phosphatase activity, and prostaglandin E2 production are not significantly altered in the presence of PEMF (7, 8). Thus, it seems unlikely that the clinical success of PEMF is entirely attributable to an effect on osteoblasts alone.
This has led investigators to study the effect of PEMF on other processes important during tissue repair (9). Angiogenesis, the sprouting of new blood vessels, is critical for successful fracture healing (10, 11), but the effects of PEMF on angiogenesis are not well understood (12). In this study, we examined the effects of PEMF on angiogenesis and its primary component, the endothelial cell.
MATERIALS AND METHODS
Cell culture
Human umbilical vein endothelial cells (HUVECs) (Clonetics, East Rutherford, NJ) were cultured in endothelial basal medium (EBM)-2 supplemented with EGM-2MV and studied at passages 4–7. Fibroblasts were harvested from newborn foreskin specimens (13) and osteoblasts from fetal rat calvaria (10). Both fibroblasts and osteoblasts were cultured in Dulbecco's modified Eagle's medium (DMEM) supplemented with 10% fetal bovine serum (FBS) and 100 µg/ml penicillin G, 50 µg/ml streptomycin, and 0.25 µg/ml amphotericin B.
Exposure to PEMF
PEMF were generated by a bone-healing device (EBI, Parsippany, NJ) delivering uniform time-varying fields. Fields consisted of asymmetric 4.5 msec pulses repeated at 15 Hz, with a magnetic flux density rising from 0 to 12 G in 200 µsec and returning to 0 G in 25 µsec. PEMF generators were placed inside identical incubators but were turned on only in the test incubator. Extraneous 50-Hz magnetic fields within each incubator were <2 mG. Custom designed cages surrounded with the same configuration were used for the in vivo experiments.
In vitro angiogenesis assay
A microcarrier (MC) in vitro angiogenesis assay was performed as described previously (14). HUVECs were added to a suspension of MCs (Cytodex 3, Amersham Biosciences, Piscataway, NJ) and cultured until confluent. Fibrin gels were prepared by dissolving fibrinogen (Sigma, St. Louis, MO) in PBS (2.5 mg/ml) along with 200 U/ml of aprotinin to prevent excessive fibrinolysis. Confluent HUVEC-seeded MCs were added to each well, and polymerization was achieved at 1 h by adding thrombin (0.625 U/ml). Gels were cultured in the presence or absence of PEMF for 7–10 days. The degree of angiogenesis was quantified by two blinded observers assessing 50 MCs at random and counting 1) the number of MCs with tubules greater than one, two, or three MC diameters and 2) the exact number of tubules on each MC.
(page number not for citation purposes)
Proliferation assay
HUVECs (1×105) seeded onto six-well plates were cultured for 24 h with EBM+1% FBS (starved media). The media was then changed to fully supplemented media, at which time cultures were separated into their respective incubators for an additional 24 h. A 24 h proliferation assay was performed by the addition of 5 µCi of radioactive thymidine [3H] 3 h before the completion of the assay. Cells were washed with PBS ×3 and 10% trichloroacetic acid ×3, followed by the addition of 2 mL of 1 N NaOH for 30 min and neutralizations with 2 mL 1 N HCl. Independent cell cultures were used for each experiment (
n=6), run in triplicate, and evaluated using a scintillation counter. A subset of HUVEC cultures (
n=3) were trypsinized, and the number of cells/well was counted manually with a hematocytometer after 24 h exposure to PEMF.
For fibroblast and osteoblast proliferation studies, 1 × 105 cells were seeded onto six-well plates in starved DMEM (with 1% FBS) and replaced with media collected from HUVEC cultures after 24 h pulsed electromagnetic field (PEMF) exposure. After 24 h, proliferation was measured by thymidine incorporation as above.
Migration assay
Migration was studied using a modified transwell assay. HUVECs and fibroblasts in starvation media (EBM or DEMM+1% FBS) were seeded onto Chemotx filters (5.7 mm, 8 µm pore size, Neuro Probe, Gaithersburg, MD). Media (either stock EBM, DMEM, or collected from HUVEC cultures after 24 h PEMF exposure) was added to the lower chamber. After 24 h incubation, nonmigrating cells were completely wiped from the top surface of the membrane, and migrating cells adherent to the underside of the filter were quantified using the nuclear dye DAPI (Vector Labs, Burlingame, CA) and fluorescent microscopy.
Media denaturing experiments
Media from HUVEC cultures in the absence or presence of PEMF was collected as donor media (conditioned media). In addition, PEMF and normal-conditioned media were heated at 100°C for 20 min and immediately cooled on ice for 20 min. The denatured media was resupplemented with EGM-2MV to replace essential growth factors that were also denatured and then similarly used as growth media for a HUVEC proliferation assay. Results were normalized to the thymidine incorporation observed in cultures receiving media harvested from HUVECs not exposed to PEMF (
n=6).
Prostaglandin synthesis inhibition
A 48 h thymidine incorporation assay was performed in the presence of indomethacin (Calbiochem, San Diego, CA), a phospholipase A2 inhibitor (15), at a concentration of 4 µg/ml, previously shown to completely block prostaglandin synthesis in vitro (
n=4) (16).
(page number not for citation purposes)
Vascular endothelial growth factor (VEGF) ELISA and Northern blot analysis
A mouse VEGF sandwich enzyme immunoassay (R&D Systems, Minneapolis, MN) was used to measure the quantity of VEGF (165-amino acid isoform) in media from PEMF and control cultures (
n=4; run in triplicate). For Northern blot analysis, total cellular RNA was extracted by cell lysis (TRIzol). RNA (20µg) was separated on a 1% agarose containing 2.0 M formaldehyde and transferred to a Brightstar-Plus nylon blotting membrane (Ambion, Woodward, TX) via Turbo Blot downward transfer system. RNA was crosslinked via the UV Stratalinker 1800 (Stratagene, La Jolla, CA) and hybridized with VEGF and 18S cDNA probes labeled with P32-dCTP (Amersham Biosciences, Piscataway, NJ) (10). Band densitometry was performed using Kodak (Rochester, NY) 1D.
VEGF blocking assays
PEMF- and control-conditioned media were used for a 48 h HUVEC proliferation assay and supplemented with either 0.1 mg/ml of anti-human VEGF antibody or 50 ng/ml of recombinant human VEGF R2(KDR)/Fc chimera (R&D Systems), concentrations previously shown to eliminate soluble VEGF activity (
n=4; run in triplicate) (17).
Angiogenic protein screening
PEMF and control conditioned media was harvested after 48 h of incubation and analyzed via a sandwich ELISA assay (SearchLight Angiogenesis Array, Pierce Technologies, Boston MA). Media samples (50 µl) were incubated for 1 h in ELISA coated with antibodies to angiogenic proteins, including tissue inhibitor of matrix metalloproteinase-1 (TIMP-1), angiopoietin-2 (Ang-2), platelet-derived growth factor (PDGF), thrombopoietin (TPO), keratinocyte growth factor (KGF), hepatocyte growth factor (HGF), and epidermal growth factor (EGF). Total concentrations (pg/ml) were determined through chemiluminescent signaling. All experiments (
n=3) were done in triplicate.
FGF-2 ELISA and Northern blot
A mouse FGF-2 sandwich enzyme immunoassay (R&D Systems) was used to measure the quantity of FGF-2 in media from PEMF and control cultures (
n=4; experiments run in triplicate). For Northern blot analysis (
n=3), total cellular RNA was extracted by cell lysis (TRIzol). RNA (50 µg) was separated on a 1% agarose containing 2.0 M formaldehyde and transferred to a Brightstar-Plus nylon blotting membrane (Ambion) via Turbo Blot downward transfer system. RNA was crosslinked via the UV Stratalinker 1800 (Stratagene) and hybridized with FGF-2 and 18S cDNA probes labeled with P32-dCTP (Amersham Biosciences) (10). Band densitometry was performed using Kodak 1D.
FGF-2 blocking assays
FGF-2 neutralizing antibody (donated by Dr. David A. Moscatelli, New York, NY) was added to cultures also receiving either PEMF- or control-conditioned media (18). The FGF-2 antibody was added each time the media was changed, and a thymidine incorporation assay was performed after 48 h (
n=6; each experiment was run in triplicate).
(page number not for citation purposes)
In vivo Matrigel plug assay
All experiments were performed in full accordance with the NYU Medical Center Institutional Animal Care and Use Committee.
Tie2/lacZ mice (10–16 wk old; Jackson Laboratory, Bar Harbor, ME) received a s.c. injection with Matrigel supplemented with basic-FGF-2 (25 ng). Mice were housed in either control cages (
n=8) or in cages that delivered PEMF (
n=10) consecutively for 8 h per day. Matrigel samples were snap frozen or fixed in 4% paraformaldehyde, and tissue sections were stained histochemically with x-gal solution overnight at 4°C or immunohistochemically with rat anti-mouse CD31 (Becton-Dickinson, Franklin Lakes, NH) and Alexa-Flour goat anti-rat secondary antibody (Molecular Probes, Eugen, OR). The number of nuclei contained per high power field (hpf) of Matrigel was counted in 20 random fields by two blinded observers. For ELISA analysis, matrigel plugs were harvested and submerged in T-PER Extraction Reagent (Pierce/Perbio, Inc.) with 100 µl/ml protease inhibitor added, mechanically homogenized, and centrifuged. Supernatent was removed and assayed using the ELISA protocol described previously for FGF-2. Similar sandwich enzyme immunoassay kits (R&D Systems) were used to assay TPO, Ang-2, and EGF (
n=4; experiments run in triplicate).
Statistical analysis
Statistical analysis was calculated based on a two-tailed
t test, and all data are presented as mean ± SE.
P < 0.05 was considered statistically significant.
PEMF induces endothelial tubule formation
The gelatin microcarrier assay is a well-established in vitro model of angiogenesis and quantifies the ability of endothelial cells to sprout from a single focus (14). HUVECs grown on MCs in the monstrate a substantial increase in e total fraction of MCs with a tubule tal number of tubules on each mione or two diameters was seen diameters (6/50 vs. 0/50;
P<0.05). Exposure to PEMF also led to a tubules per microcarrier (2.25±0.45 vs. 1.00±0.25;
P
PEMF stimulates endothelial proliferation
Thymidine incorporation established that HUVECs exposed to PEMF demonstrated enhanced proliferation compared with controls (9.2×104 vs. 3.5×104 cpm;
Pincrease in proliferation correlated with an increase in absolu103 cells/well in PEMF vs. 117±9×103 cells/well in controls) over the 24 h course of the experiments. Fibroblast and osteoblast cell lines, under identical conditions of PEMF exposure, did not exhibit any change in thymidine incorporation or cell number (
P=0.23 and
P=0.29, respectively).
(page number not for citation purposes)
PEMF releases the soluble pro-angiogenic protein FGF-2
Media harvested from HUVECs cultured in PEMF (PEMF-conditioned media) increased proliferation of HUVECs not directly exposed to PEMF, suggesting a soluble factor was responsible. The average HUVEC response to PEMF-conditioned media was twofold greater than HUVECs given media from control cells not exposed to PEMF (222.0±2.3%;
P<0.01). The addition of a cyclooxygenase inhibitor (indomethacin) was unable to block PEMF-induced stimulation (206.0±2.7%;
P<0.01), suggesting that arachadonic acid metabolites were not involved. In contrast, heat denaturing eliminated the stimulatory effects of PEMF-conditioned media on HUVECsmonstrating that a soluble protein was responsible for the
The most likely candidate responsible for pro-angiogenic effect was VEGF, a potent vascular mitogen (19). However, no differences were observed in VEGF-A mRNA or protein levels within PEMF cultures when compared with controls (mean intensity 167.57 vs. 172.23, 51.25±4.98 pg/ml vs. 50.79±3.78 pg/ml, respectively;
Pthat VEGF signaling was not involved, proliferation assaysed in the presence of anti-VEGF antibody or recombinant VEGF-receptor 2 (KDR)/Fc chimera, both potent blockers of soluble VEGF activity. HUVEC proliferation in response to PEMF was unchanged in conditioned-me
Because VEGF is only one of many potential angiogenic factors, angiogenic protein screening of PEMF conditioned media was performed. Protein concentrations for tissue inhibitor of matrix metalloproteinase-1 (TIMP-2), platelet-derived growth factor (PDGF), and hepatocyte growth factor (HGF) were not significantly altered in PEMF conditions vs. controls (data not shown). In ound by ELISA to be increased fivefold by exposure to PEMF lot analysis also revealed an increase in FGF-2 mRNA in cultures ean intensity 975635 vs. 651316;
PFGF-2 neutralizing antibody inhibited the stimulatory effects ofbut did not return it to base Additional proteins with smaller significant elevations were Ang-2, TPO, and EGF: (2320.3±1128.4 vs. 3323.8±1168.7 pg/ml;
P<0.05), (46.7±4.3 vs. 133.1±51.4 pg/ml;
P<0.05), and (4.8±1.3 vs. 7.1±0.4 pg/ml;
P<0.05), respectively (data not shown).
Conditioned media stimulates proliferation in fibroblasts but not osteoblasts
Under direct stimulation with PEMF, HUVECs proliferated exponentially and released significant amounts of FGF-2. However, fibroblast and osteoblast proliferation did not increase appreciably after PEMF exposure. To determine whether paracrine FGF-2 signaling occurred from HUVECs to parenchymal tissues, we studied fibroblast and osteoblast proliferation under the influence of media collected from HUVEC cultures after 24 h PEMF exposure. Using the same thymidine assay described previously, 24 h of exposure to conditioned media resulted in a significant (>100%) increase in fibroblast growth when composteoblast proliferation did not change significantly under the same
(page number not for citation purposes)
Conditioned media stimulates fibroblast and HUVEC migration
To further confirm the importance of FGF-2 signaling from HUVECs and examine functional cell changes induced by protein release, we studied fibroblast and HUVEC migration using PEMF-conditioned HUVEC media as a chemotactic agent. The migratory populations of both Cs more than doubled under the influence of PEMF-conditioned media
PEMF stimulates in vivo angiogenesis
Having demonstrated that PEMF has a potent effect on endothelial cells in vitro, we examined whether PEMF was able to stimulate angiogenesis in vivo
. Matrigel is a soluble basement membrane preparation, and when implanted s.c. supports vascular ingrowth. Matrigel was injected s.c. into
tie2/lacZ transgenic mice that were housed in cages emitting PEMF for 8 h a day or control cages. After 3, 10, and 14 days, there was significantly greater vascular ingrowth into the matrix in PEMF-treated animals, confirmed by staining specific for endothelial markers CD31 and Tie-2. PEMF increased the vascular ingrowth more than twofold by day 3 (13.3±0.41 vs. 5.8±0.28 cells/hpf;
P<0.01). This increase in vascular ingrowth persisted through days 10 and 14 (16.6±0.49 vs 12.6±0.43 cells/hpf;
P<0.01, and 19.4±0.55 vs. 14.8±0.40 cells/hpf;
P<0.01, respectivelLISA confirmed a twofold increase in FGF-2 in PEMF-treated matrigel, but demfactors TPO, Ang-2, and EGF (data not shown).
In this study, we demonstrate that PEMF stimulates processes critical for angiogenesis. The delivery of PEMF at low doses, identical to that currently in clinical use, significantly increased endothelial cell proliferation and tubulization, processes important for vessel formation. The ability of PEMF to increase cellular proliferation was unique to endothelial cells while the addition of media from conditioned HUVECs to both fibroblast and HUVEC cultures increased proliferation and migration. This suggests that endothelial cells are the primary target for PEMF stimulation, releasing protein in a paracrine fashion to induce changes in neighboring cells and up-regulate angiogenesis. However, both direct stimulation and conditioned media studies revealed no significant change in osteoblast proliferation. Thus, the ability of PEMF to enhance the healing of complicated fractures is likely the result of increased vascularity rather than a direct effect on osteogenesis as previously believed.
While VEGF is the most ubiquitous mediator of angiogenesis, it was not responsible for the angiogenic effect of PEMF in these experiments. Angiogenic protein screening demonstrated a fivefold increase in FGF-2, a well-described angiogenic mediator. While the addition of an FGF-2 neutralizing antibody reduced PEMF stimulation of endothelial cells, proliferation did not return completely to baseline. It is therefore possible that PEMF does not simply act through the up-regulation of a single agent (i.e., FGF-2), but involves the coordinated release of other angiogenic proteins or cytokines. However, in vivo, we only were able to demonstrate significant increases in FGF-2. Thus, it seems likely that FGF-2 signaling is the predominant mechanism and these cytokine changes are secondary. It is interesting to note that the in vitro potency of PEMF to increase endothelial cell proliferation was comparable to that of high doses of VEGF or FGF, suggesting that this phenomenon is of true biologic relevance in vivo (20).
(page number not for citation purposes)
To support this, we examined the effect of PEMF on in vivo angiogenesis. Using the well-established Matrigel assay, we demonstrated that PEMF was able to significantly increase angiogenesis in vivo. Recent evidence suggests that blood vessels in the adult may result from either expansion of existing endothelial cells or the recruitment of bone marrow-derived endothelial progenitor cells (EPCs) (21, 22). Although we did not directly assess the effects of PEMF on bone marrow derived EPCs, our in vitro data on fully differentiated endothelial cells leads us to suspect that the effects of PEMF are directed toward pre-existing endothelial cells.
If PEMF is able to augment angiogenesis, its clinical utility may extend well beyond its current role in bone healing. One obvious application is in the field of
therapeutic angiogenesis, defined as the artificial manipulation of blood vessel growth for the treatment of ischemic conditions. The majority of existing techniques for therapeutic angiogenesis are based on the delivery of single pro-angiogenic cytokines or the supplementation of vascular stem cells (21). Agents such as VEGF or FGF have shown promise in animal models, but clinical trials have been disappointing (23). Furthermore, difficulties related to immunogenicity, dosing, and means of delivery have limited the widespread clinical impact of these modalities. PEMF may offer distinct advantages as a noninvasive and targeted modality, which is able to release several growth factors to achieve therapeutic angiogenesis. Moreover, because PEMF utilizes commonly available, clinically approved technology, it may have rapid applicability in the treatment of ischemic conditions (24). Data from this study provide a rational basis for use in these conditions.
The finding that PEMF was able to stimulate endothelial cell kinetics raises important questions regarding the relationship between PEMF and carcinogenesis. A number of epidemiological studies have suggested a link between electromagnetic fields and malignancies, including breast cancer, brain cancer, and leukemia (25), but the precise mechanism, if any, remains unknown. Although there are multiple papers confirming that electromagnetic fields are not directly mutagenic or carcinogenic, none have examined the possibility that electromagnetic fields may promote tumor progression once malignant transformation has occurred. Because angiogenesis is believed to be essential for tumor growth, spread, and eventual clinical disease, the present study suggests that the link between electromagnetic fields and cancer may be through increased angiogenesis. Epidemiological studies suggest that exposure to PEMF (i.e., high-tension power lines) at a wide range of frequencies can be correlated with an increased risk of cancer (26). However, the direct comparison to the field strength used in this study is difficult given the wide amplitude window produced by pulsed delivery. Although clinical data suggest that PEMF is safe, the possibility that electromagnetic fields are not themselves carcinogenic but promote tumor progression via increased angiogenesis warrants further investigation.
In conclusion, although PEMF has been used for years by clinicians to supplement bone healing, its precise mechanism of action has not been determined. Our data provide evidence to support the concept that PEMF acts by promoting angiogenesis through the coordinated release of FGF-2 and to a lesser extent several other vascular growth factors (Ang-2, TPO, and EGF). This suggests that PEMF may facilitate healing by augmenting the interaction between osteogenesis and blood vessel growth. This finding not only elucidates a novel mechanism for PEMF action, but also suggests extended applications for PEMF in the treatment of ischemic disease and a potential linkage between electromagnetic fields and tumor biology.
(page number not for citation purposes)
This work was funded by the Juvenile Diabetes Research Foundation (O.T.) and the Sarnoff Endowment for Cardiovascular Research (G.G.). We wish to thank David A. Moscatelli for his input and Marie Frisone for her assistance in preparation of the manuscript.
1. Bassett, C. A., Pawluk, R. J., and Pilla, A. A. (1974) Augmentation of bone repair by
inductively coupled electromagnetic fields.
Science 184, 575–577
2. Ryaby, J. T. (1998) Clinical effects of electromagnetic and electric fields on fracture
healing.
Clin. Orthop. 355S, S205–S215
3. Scott, G., and King, J. B. (1994) A prospective, double-blind trial of electrical capacitive
coupling in the treatment of non-union of long bones.
J. Bone Joint Surg. Am. 76, 820–826
4. Sharrard, W. J. (1990) A double-blind trial of pulsed electromagnetic fields for delayed
union of tibial fractures.
J. Bone Joint Surg. Br. 72, 347–355
5. Bostrom, M. P., et al. (1995) Immunolocalization and expression of bone morphogenetic
proteins 2 and 4 in fracture healing.
J. Orthop. Res. 13, 357–367
6. Bodamyali, T., et al. (1998) Pulsed electromagnetic fields simultaneously induce
osteogenesis and up-regulate transcription of bone morphogenetic proteins 2 and 4 in rat
osteoblasts in vitro.
Biochem. Biophys. Res. Commun. 250, 458–461
7. Guerkov, H.H. et al. (2001) Pulsed electromagnetic fields increase growth factor release by
nonunion cells.
Clin. Orthop. 384, 265–279
8. Aaron, R. K., Wang, S., and Ciombor, D. M. (2002) Up-regulation of basal TGF-beta1
levels by EMF coincident with chondrogenesis–implications for skeletal repair and tissue
engineering.
J. Orthop. Res. 20, 233–240
9. Jasti, A. C., et al. (2001) Effect of a wound healing electromagnetic field on inflammatory
cytokine gene expression in rats.
Biomed. Sci. Instrum. 37, 209–214
10. Steinbrech, D. S., et al. (2000) VEGF expression in an osteoblast-like cell line is regulated
by a hypoxia response mechanism.
Am. J. Physiol. Cell Physiol. 278, C853–C860
11. Donski, P. K., Carwell, G. R., and Sharzer, L. A. (1979) Growth in revascularized bone
grafts in young puppies.
Plast. Reconstr. Surg. 64, 239–243
12. Yen-Patton, G. P., Patton, W. F., Beer, D. M., and Jacobson, B. S. (1988) Endothelial cell
response to pulsed electromagnetic fields: stimulation of growth rate and angiogenesis in
vitro.
J. Cell. Physiol. 134, 37–46
(page number not for citation purposes)
13. Freshney, R. I. (2000) Primary Cell Culture, in
Culture of Animal Cells: A Manual of Basic
Technique, 4th ed, 149–175, Wiley-Liss, New York
14. Nehls, V., and Drenckhahn, D. (1995) A novel, microcarrier-based in vitro assay for rapid
and reliable quantification of three-dimensional cell migration and angiogenesis.
Microvasc.
Res. 50, 311–322
15. Fitzpatrick, F. A., and Murphy, R. C. (1988) Cytochrome P-450 metabolism of arachidonic
acid: formation and biological actions of "epoxygenase"-derived eicosanoids.
Pharmacol.
Rev. 40, 229–241
16. Brighton, C. T., Wang, W., Seldes, R., Zhang, G., and Pollack, S. R. (2001) Signal
transduction in electrically stimulated bone cells.
J. Bone Joint Surg. Am. 83-A, 1514–1523
17. Millauer, B., et al. (1993) High affinity VEGF binding and developmental expression
suggest Flk-1 as a major regulator of vasculogenesis and angiogenesis.
Cell 72, 835–846
18. Sato, Y., and Rifkin, D. B. (1988) Autocrine activities of basic fibroblast growth factor:
regulation of endothelial cell movement, plasminogen activator synthesis, and DNA
synthesis.
J. Cell Biol. 107, 1199–1205
19. Losordo, D. W., et al. (1998) Gene therapy for myocardial angiogenesis: initial clinical
results with direct myocardial injection of phVEGF165 as sole therapy for myocardial
ischemia.
Circulation 98, 2800–2804
20. Bernatchez, P. N., Rollin, S., Soker, S., and Sirois, M. G. (2002) Relative effects of VEGF-
A and VEGF-C on endothelial cell proliferation, migration and PAF synthesis: Role of
neuropilin-1.
J. Cell. Biochem. 85, 629–639
21. Isner, J. M., and Asahara, T. (1999) Angiogenesis and vasculogenesis as therapeutic
strategies for postnatal neovascularization.
J. Clin. Invest. 103, 1231–1236
22. Tepper, O. M., et al. (2002) Human endothelial progenitor cells from type II diabetics
exhibit impaired proliferation, adhesion, and incorporation into vascular structures.
Circulation 106, 2781–2786
23. Carmeliet, P. (2000) VEGF gene therapy: stimulating angiogenesis or angioma-genesis?
Nat. Med. 6, 1102–1103
24. Kanno, S., et al. (1999) Establishment of a simple and practical procedure applicable to
therapeutic angiogenesis.
Circulation 99, 2682–2687
25. Stix, G. (1998) Closing the book. Are power-line fields a dead issue?
Sci. Am. 278, 33–34
26. Savitz, D. A., and Calle, E. E. (1987) Leukemia and occupational exposure to
electromagnetic fields: review of epidemiologic surveys.
J. Occup. Med. 29, 47–51
Received November 9, 2003; accepted May 4, 2004.
(page number not for citation purposes)
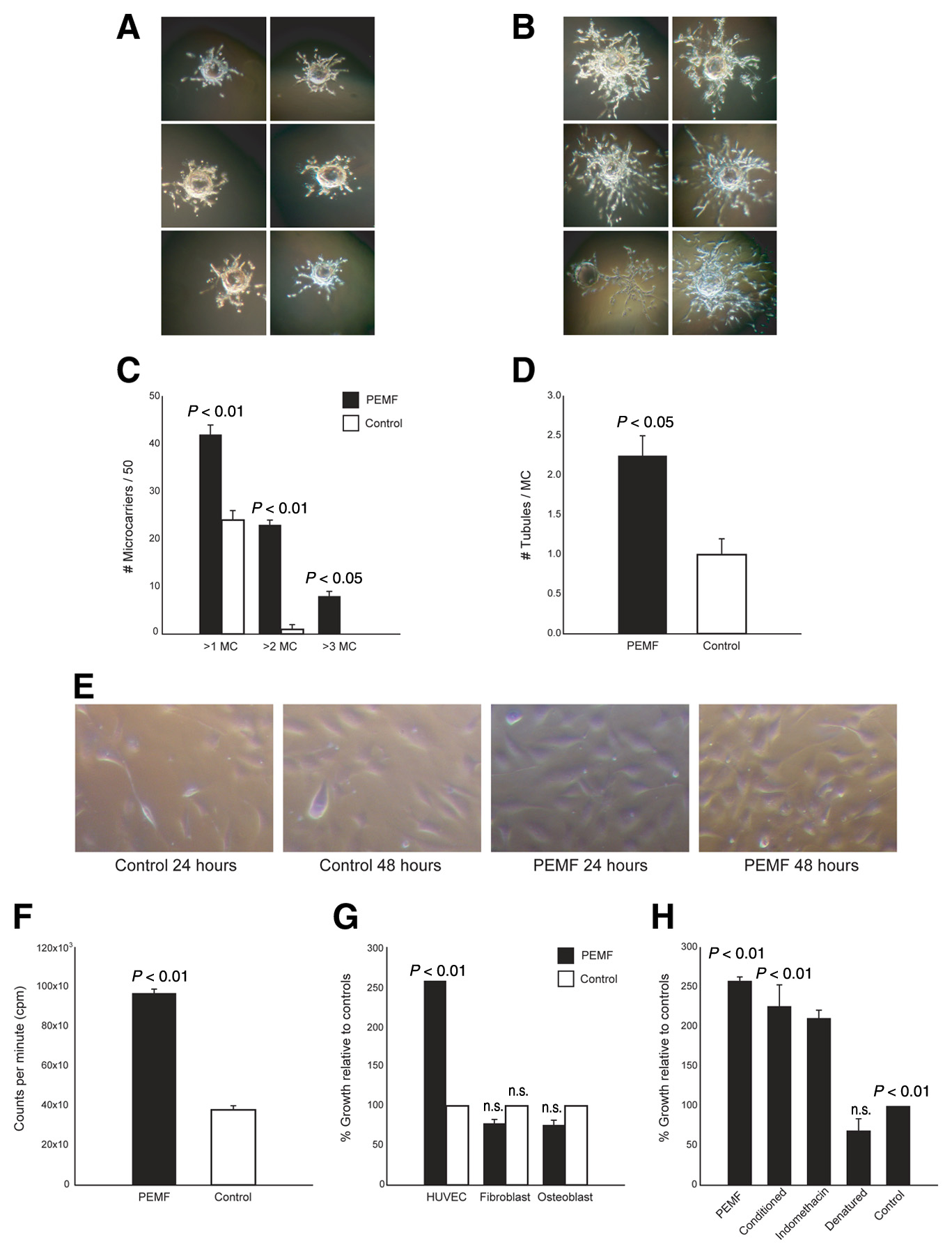
(page number not for citation purposes)
Figure 1. PEMF stimulates 3-D angiogenesis in vitro. A 3-D angiogenesis assay was performed on HUVECs grown on
gelatin microcarriers and embedded in a fibrin gel. Representative pictures 7 days after HUVEC-seeded microcarriers were
cultured in normal conditions (A) or PEMF (B), demonstrating increased tubulization of PEMF. C) Fifty microcarriers
were chosen at random, and the number of microcarriers exhibiting tubulization of greater than 1 diameter (>1MC), 2
diameters (>2MC), or 3 diameters (>3MC) was quantified. D) Microcarriers were also assessed for the number of tubules
present on each microcarrier. The extent of proliferation of HUVECs over a 48-h period was examined by light
microscopy (E) and quantified by thymidine incorporation (F) and revealed that PEMF significantly augmented the
proliferation of HUVECs (P<0.01) but had no effect on osteoblasts or fibroblasts (G). H) Media cultured in PEMF was
able to enhance the proliferation of HUVECs, but denaturing the media ablated this effect. HUVEC proliferation in PEMF
was not inhibited by the addition of indomethacin, a prostaglandin (PGE2) synthesis inhibitor.
(page number not for citation purposes)
(page number not for citation purposes)
Fig. 2 (cont)
Figure 2. PEMF stimulates the release of FGF-2 but not VEGF by HUVECs. A) Northern blot analysis demonstrated
no change in VEGF mRNA when HUVECs were grown in PEMF or control conditions. B) VEGF ELISA revealed no
differences in VEGF protein production by HUVECs exposed to PEMF. C) The presence of recombinant VEGF R2/Fc
chimera or anti-VEGF antibody was unable to block the response of HUVEC proliferation to PEMF. In contrast, FGF-2
production was found to be twofold greater in PEMF conditions. D) FGF-2 ELISA verified that PEMF stimulated a
threefold increase in HUVEC production of FGF-2 protein. E) Northern blot analysis demonstrated an increased degree of
FGF-2 transcription in response to PEMF, normalized using GAPDH. F) The addition of FGF-2 neutralizing antibody
significantly reduced the degree of stimulation in response to PEMF. Control cultures exhibited no changes in proliferation
in response to the antibody. G) Media collected from PEMF cultures was able to induce significant (>100% above
baseline) proliferation in HUVECs and fibroblasts but not osteoblasts. H) Migration of fibroblasts and HUVECs in
response to media collected form PEMF-conditioned HUVEC cultures was threefold greater in both cell types compared
with cells stimulated with unconditioned media.
(page number not for citation purposes)
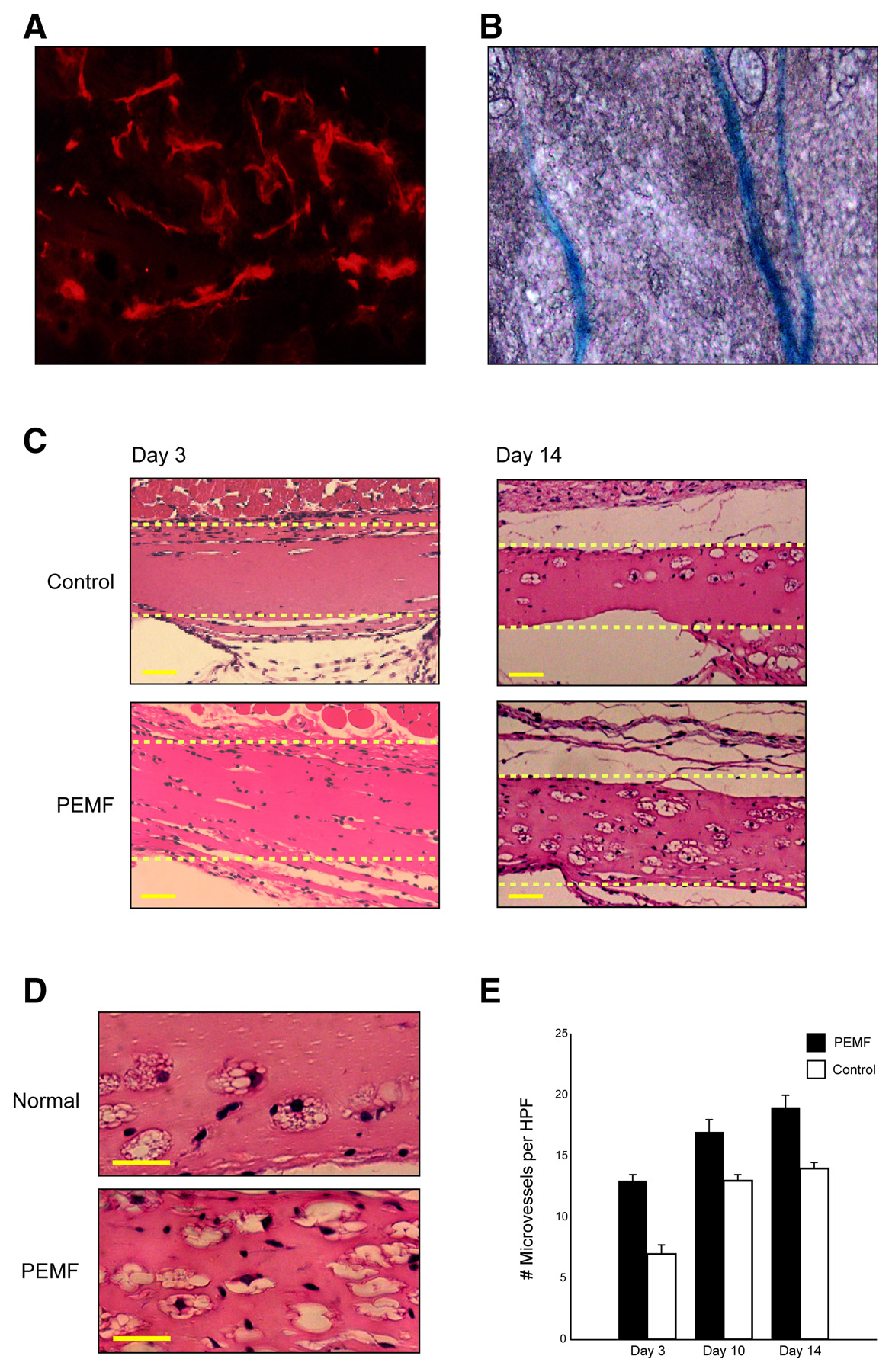
(page number not for citation purposes)
Figure 3. PEMF promotes angiogenesis in an in vivo Matrigel plug assay. A, B) Vascular ingrowth
within Martigel 2 wk after implantation was confirmed by staining for CD31 and Tie2. C) Representative
pictures of Matrigel (dotted box) from mice in control and PEMF cages demonstrate that exposure to
PEMF led to an increase in the degree of vascular ingrowth relative to controls. (Scale bars represent
25µm) D) High-power views of a representative section of Matrigel from control mice (top panel) and
PEMF-exposed mice (bottom panel) are depicted. E) Quantification of cells within the Matrigel
demonstrated a significant PEMF stimulation of vascular ingrowth at days 3, 10, and 14 (scale bars
represent 25 µm).
(page number not for citation purposes)
Source: http://sthetica.pl/wp-content/uploads/2015/07/artyk-angiogenesis.pdf
CAPRIS GIUSTINOPOLI Aldo Cherini – Autoedizione 1992 Recupero da Ventura 28-09-2001 La Gorgona e il Sole, stemmi di Capodistria con pari valore rappresentativo, sono d'oro in campo azzurro e anche la bandiera municipale porta gli stessi colori: ecco perché "Oro e Colori che una schiera infinita di cittadini ha tenuto alti nel corso della storia patria
Perceived Risks Incurred Along With Genetic Engineering's Alluring Benefits New approaches to the practice of medicine and to food production have arrived on the technolo-gical scene as a consequence of the knowledge gained through modern genome sequencing. Now Scientists and genetic engineers can modify and manipulate the fundamental genetic code of cer-tain crops and animals to benefit mankind. Although the stated goals of genetic engineering and genetic modification are noble, these activities are not without associated risks and drawbacks, which apparently have received much less attention. The discussion below is an attempt to reme-dy that oversight by discussing both aspects in one gulp in a form that is easy to understand, without hype, with the venom removed and the invective wording toned down (from how it was originally stated elsewhere in many cases). We eschew being extreme in portraying either view; otherwise, adversaries would dismiss it out-of-hand as not being worthy to even consider further. In compiling the list of risks (and benefits) here below, we rely only on information and facts provided by internationally recognized scientific journals, nationally recognized newspapers, and three of the Public Broadcasting System's (PBS) NOVA series [1]-[3]. Each of these particular sources is well known for thoroughly crosschecking its supporting facts and, moreover, is avail-able from most U.S. public libraries for further confirmation. We specifically avoided visiting or viewing Web sites, pro or con (such as those of the USDA, FDA, EPA, The Foundation for Eco-nomic Trends, Friends of the Earth, International Green Peace, or the Earth Liberation Front) because information posted on the Web, in general, is notorious for being self-serving (by dis-seminating biases interspersed with truth), ephemeral (since it can vanish), and time-varying. Our aim here is not to convert or dissuade any existing views away from the current U.S. policy of encouraging progress in genetic engineering/genetic modification because the apparent con-sensus is that it is important for the long-term welfare of the U.S. and the world, both scientific-cally and economically, to continue on this course. The objective here is merely to delineate the benefits (as perceived by its advocates) and the drawbacks (as perceived by its opponents) of Genetically Modified Organisms (GMO), all in one place. The worries of the GMO critics appear to all pertain, at a high level, to one or more of the following four questions: